INTRODUCTION
Metabolic disorders such as obesity and type 2 diabetes (T2DM) are leading causes of morbidity and mortality for the world’s growing population (Rodgers et al., 2005; Deshpande et al., 2008; Pfluger et al., 2008). In particular, T2DM is the most common, accounting for 90% of patients in these categories (Wang et al., 2017). Therefore, the effective control of blood glucose is the key to preventing adverse impacts on the quality of life for type 2 diabetes patients (Shafiee et al., 2012). T2DM is caused by resistance to the action of insulin combined with a deficiency in insulin secretion; recently, functional foods and their bioactive compounds have been found to be useful as complementary treatments for T2DM (Patel et al., 2012).
Bitter melon (Momordica charantia L.; MC), or bitter gourd, is a popular plant used for its anti-diabetic, anti-cancer, anti-inflammatory and cholesterol-lowering effects among the indigenous populations of Asia, South America, India, the Caribbean and East Africa. In Chinese and Indian traditional medicine, bitter melon has typically been used to treat hypoglycemia and diabetes (Tan et al., 2008).
In previous studies, this plant was reported to have a preventive effect on the development of hyperglycemia in KK-Ay mice, which spontaneously develop T2DM (Alam et al., 2015). It was expected from these results that bitter melon would exhibit anti-diabetic effects in other diabetic animal models with insulin resistance (Efird et al., 2014). The physiologically active substance of bitter melon has been reported to be optimized by ethanol extraction (Fulco & Sartorelli, 2008).
Recent epidemiological studies have indicated that the consumption of a high-calorie diet and the ensuing obesity are two of the principal causes of T2DM (Steyn et al., 2004). Therefore, an obesity-induced experimental model of T2DM induced by a high-calorie diet would be useful for evaluating the anti-diabetic action of bitter melon extract (Alam et al., 2015). C57BL/6 mice fed a high-fat diet (HFD) have been shown to be a representative model of obesity-induced diabetes (Hu & Malik, 2010). The HFD causes insulin resistance, and insulin sensitivity is associated with the expression of Sirtuin 1 (SIRT1) (Mayoral et al., 2015). The SIRT1 pathway regulates energy homeo- stasis at the cellular and whole-body level. It plays a central role in the regulation of glucose uptake and insulin sensitivity (Li, 2013).
Many studies have reported that the expression of SIRT1 plays a role in glucose metabolism (de Kreutzenberg et al., 2010; Li & Kazgan, 2011; Kitada & Koya, 2013). In the present study, we found that ethanol extract of bitter melon has anti-obesity and anti-diabetic effects through sirt1 in the liver tissue. Therefore, we believe that the physiologically active substance of bitter melon can be used as an anti-diabetic agent to improve glucose metabolism by SIRT1.
MATERIALS AND METHODS
Three batches (No. DHP20160217-20160219) of standardized ethanol extract of bitter melon (BME) compound were manufactured and verified by Daeho Corporation Co., Ltd. (Hwaseong, Korea). Briefly, the dried unripe fruit of bitter melon (Hamyang, Korea/Cheonryung Foods Co., Ltd.) was shattered and extracted by heating twice at 70℃ (4 h and 2 h) using 70% ethanol. The extract was then filtered and concentrated (Busung Tech, Ansung, Korea) to 15-20 degrees Brix at 65℃. The concentrated extract was spray dried (Mehyun Engineering, Anyang, Korea) at inlet temperature 175 ± 10℃ and outlet temperature 80 ± 5℃, and dextrin (49%) and lecithin (1%) were added. The extraction yield was approximately 34% (w/w).
Five-week-old male C57BL/6J mice were purchased from Central Lab Animal Inc. (Seoul, Republic of Korea). After an adaptation period of 1 week, the mice were randomly divided into four groups (n = 8-12). The normal diet group (ND) was fed a chow diet (10% kcal from fat, New Brunswick, NJ, USA, Research Diets; D12450B) or a high-fat diet (HFD) (45% kcal from fat, New Brunswick, NJ, USA, Research Diets; D12451) for 12 weeks and then was fed an HFD supplemented with BME 250 (250 mg/kg body weight/ day) or an HFD supplemented with BME 500 (500 mg/kg body weight/day) for 12 weeks. The animals were housed in a temperature-controlled environment with a 12-h light /dark cycle during the entire 12-week experimental period.
The animals’ food intake and body weight were measured weekly. At the end of the experimental period, the animals were sacrificed. Blood samples were collected from the heart using a 1-mL syringe and were drawn into a 5-mL VACUETTE tube (Greiner Bio-one, Thailand). After being centrifuged at 1,500 ×g for 10 min, the serum was stored at –80℃ until analysis. The liver was removed and weighed. Portions of mesenteric liver were immersed in 4% neutralized buffered paraformaldehyde (NBP) for histological inspection; other portions were frozen immediately in liquid nitrogen and stored at –80℃ for RNA and protein samples. The experimental protocol was approved by the Institutional Animal Care and Use Committee of Gyeongsang National University (Jinju, Republic of Korea).
Fixed liver tissue in 4% neutral paraformaldehyde was dehydrated though a graded series of ethanol (70%, 80%, 90%, 95%, and 100% absolute ethanol) for 2 h per concentration, cleared in two changes of xylene for 2 h per change and impregnated using two changes of molten paraffin wax for 2 h per change in a Leica tissue processor (Leica TP 1020, Germany). The embedded tissue was sectioned at a thickness of 5 µm with a microtome (Leica RM2235, Germany) and subjected to H&E staining. All the stained tissue sections were imaged with a virtual slide microscope (VS120 S1, Olympus BX61 VST, Hamburg, Germany) at ×20 magnification with Olyvia software.
Fixed liver tissue in 4% neutral paraformaldehyde was cryoprotected in 30% sucrose and embedded in Tissue-Tek optimal cutting temperature (OCT) compound (Sakura Fineteck USA, Inc., Torrance, CA, USA). The embedded tissue was cryosectioned at a thickness of 5 µm using a cryostat (Leica CM 1950, Germany) and subjected to oil red O staining with an Oil Red O kit (Abcam #ab 150678). All the stained tissue sections were imaged with a virtual slide microscope (VS120 S1, Olympus BX61 VST, Hamburg, Germany) at ×20 magnification with Olyvia software.
Serum samples were used to measure the content of free fatty acid (FFA), glucose, insulin, C-peptide, total cholesterol (TC) and low-density lipoprotein-cholesterol (LDL-C). These analyses were carried out at the Green Cross Reference Laboratory (Seoul, Republic of Korea). C-peptide was measured with the Lbis (R) Mouse C-peptide ELISA kit (U type) (Shibayagi Co., Ltd., Japan).
The ND was fed a chow diet or a HFD for 12 weeks and then was fed an HFD supplemented with BME 250 or an HFD supplemented with BME 500 for 12 weeks. The performed glucose tolerance tests with HFD, BME 250 and 500 mice before and after transplantation after 16 h of fasting by measuring glycemic values in peripheral blood following intraperitoneal (i.p.) injection of 4 g/kg body weight glucose in saline. Intraperitoneal glucose tolerance test (IPGTT) under stress conditions was performed by keeping the animals inside restraining boxes and injecting i.p. 2g/kg glucose in saline. Intraperitoneal insulin tolerance tests (IPITT) were performed on random-fed mice after i.p. injection of 0.75 U/kg insulin in saline.
Tissues were lysed RIPA buffer (Thermo Fisher Scientific, Waltham, MA, USA). Total protein concentrations were determined using BCA assay (PIERCE, Rockford, IL, USA). An equal volume of 4× SDS sample buffer was added, and the samples were boiled for 5 min. Equivalent amounts of total protein (20-60 μg) were separated by SDS-PAGE on 8-12% polyacrylamide gel and then transferred to nitrocellulose membrane. The membrane was blocked with 5% skim milk or 3% BSA in 0.1% Tween-20/Tris buffered saline (TBS-T). The membrane was incubated with SirT1 (1:1000, #2493, Cell Signaling Technology, MA), PPARα (1:1000, Santa Cruz Biotechnology, CA), SREBP-1(1: 1000, sc-8984, Santa Cruz Biotechnology), AMPK (1: 1000, #2532, Cell Signaling Technology), p-AMPK (1: 1000, #2535, Cell Signaling Technology), ACC (1:1000, #3676, Cell Signaling Technology), p-ACC (1:1000, #11818, Cell Signaling Technology), α-tublin (1:10000, A11126, Thermo Fisher Scientific) and β-actin (1:10000, MA5-15739, Thermo Fisher Scientific ) antibodies overnight at 4℃.
Results
The C57BL/6 mice were randomly divided into four groups (n = 8-12). The first group was fed a normal diet (ND), the second was fed a HFD, and the other two groups were fed an HFD supplemented with 250 mg BME/kg BW (BME 250) and 500 mg BME/kg BW (BME 500), respectively. The body-weight gain in the HFD group was significantly greater than that in the ND group. Notably, the body-weight gain in both the BME 250 and the BME 500 group was significantly lower than that in the HFD group (Fig. 1A). Whereas food intake in both the BME 250 and the BME 500 group was not significantly different than that in the HFD group (Fig. 1B). The weight of individual or total white adipose tissue (total WAT, epididymal, perirenal, and mesenteric) was significantly greater in the HFD group than in the ND group. The WAT weight decreased significantly after the administration of BME (Fig. 1C). BME inhibited visceral fat accumulation without affecting food intake. Liver weight decreased significantly more in the BME 250 and the BME 500 group than in the HFD group (Fig. 1D). The H&E and oil red O staining showed that BME ameliorated hepatic steatosis more in the BME 500 group than in the HFD group (Fig. 1E).
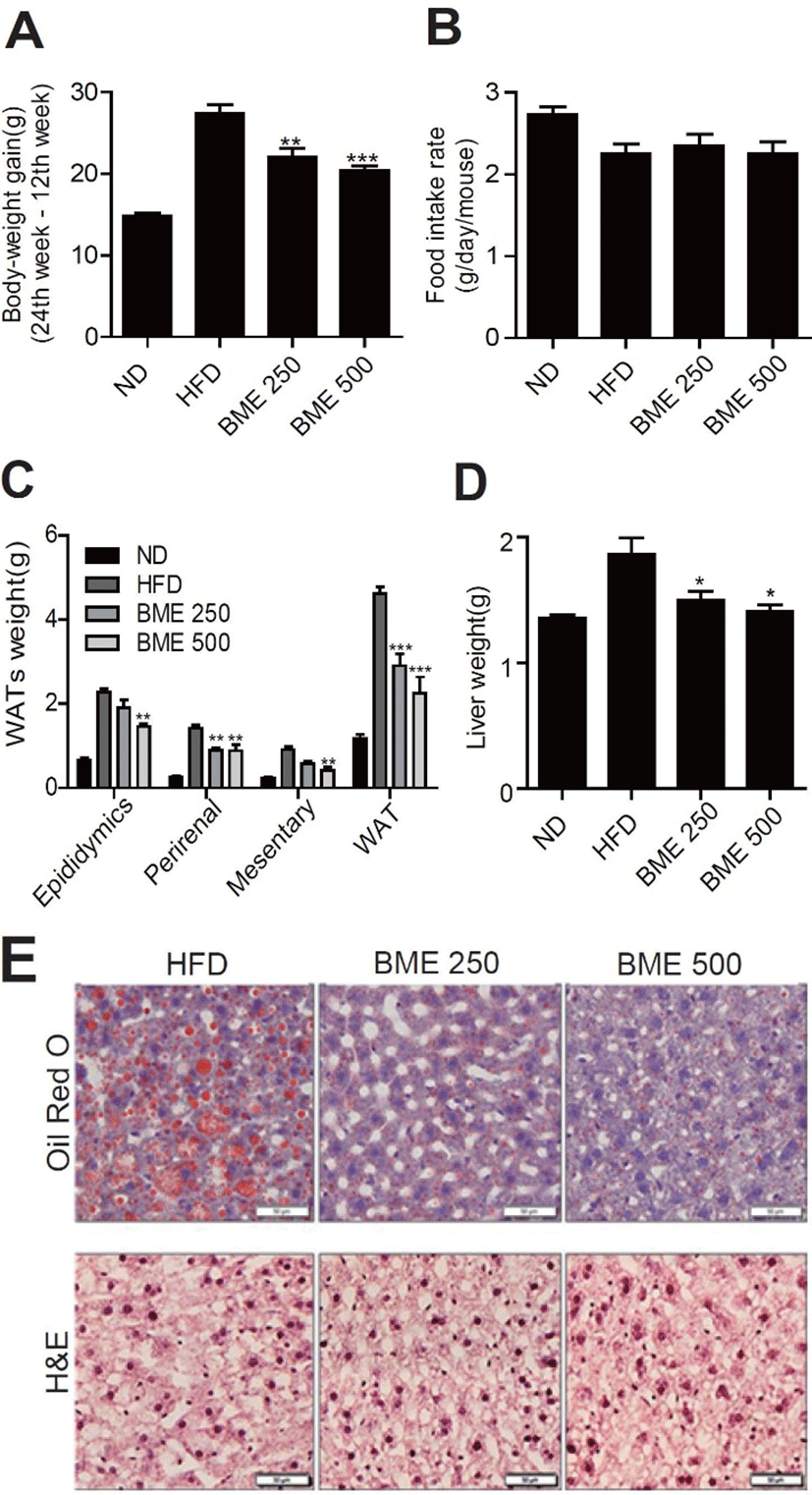
The plasma lipid (total cholesterol, LDL cholesterol, FFA) levels in the serum decreased significantly in the BME 250 and 500 groups compared with the HFD group (Fig. 2 A-C). As lipolysis, circulating free fatty acids under insulin resistance conditions, these results demonstrate that the decrease in plasma lipids may contribute at least partially to the improvement of diabetes.
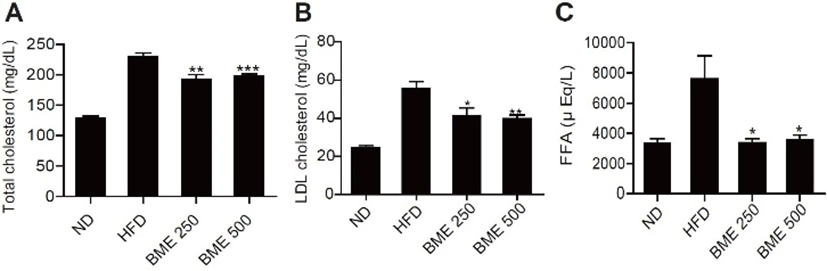
The effects of BME supplementation on fasting blood glucose levels decreased significantly more in the BME 250 and 500 groups than in the HFD group (Fig. 3A), and the plasma insulin and C-peptide levels decreased more in the BME 500 group than in the HFD group (Fig. 3 B-C).
An IPGTT performed to determine the effect of BME on glucose tolerance data showed that the glucose concentration was significantly lower in the BME 200 and BME 500 groups than in the HFD group at 30, 60 and 120 min postglucose i.p. injection (Fig. 3D). Glucose disappearance, based on IPITT results, demonstrated that BME significantly improved glucose disposal ability by enhancing insulin sensitivity (Fig. 3E).
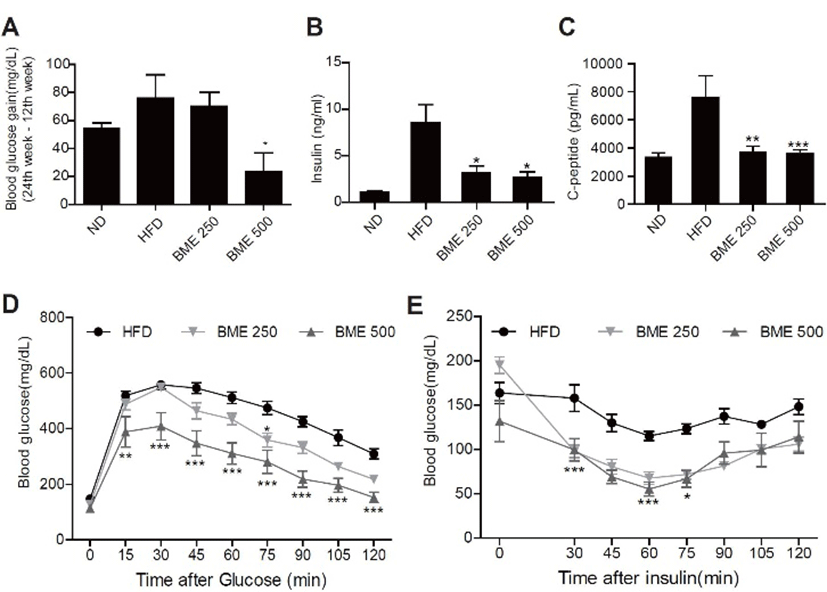
To elucidate which genes are involved in the antisteatotic and anti-diabetic effect of BME, the expressions of the genes related to hepatic lipid metabolism were measured by quantitative Western blot. The HFD group had a significantly lower expression of SIRT1 than the BME 250 and 500 groups. The BME groups showed a significant increase in the hepatic expression levels of SIRT1 (Fig. 4A). Similarly, the expression of phospho-AMPK (p-AMPK), p-ACC and ACC increased significantly in the BME 500 group (Fig. 4B). The down-signaling molecules of SIRT1, such as Foxo1 and PPARα were increased significantly and SREBP1c was decreased significant in the BME 250 and 500 group (Fig. 4C).
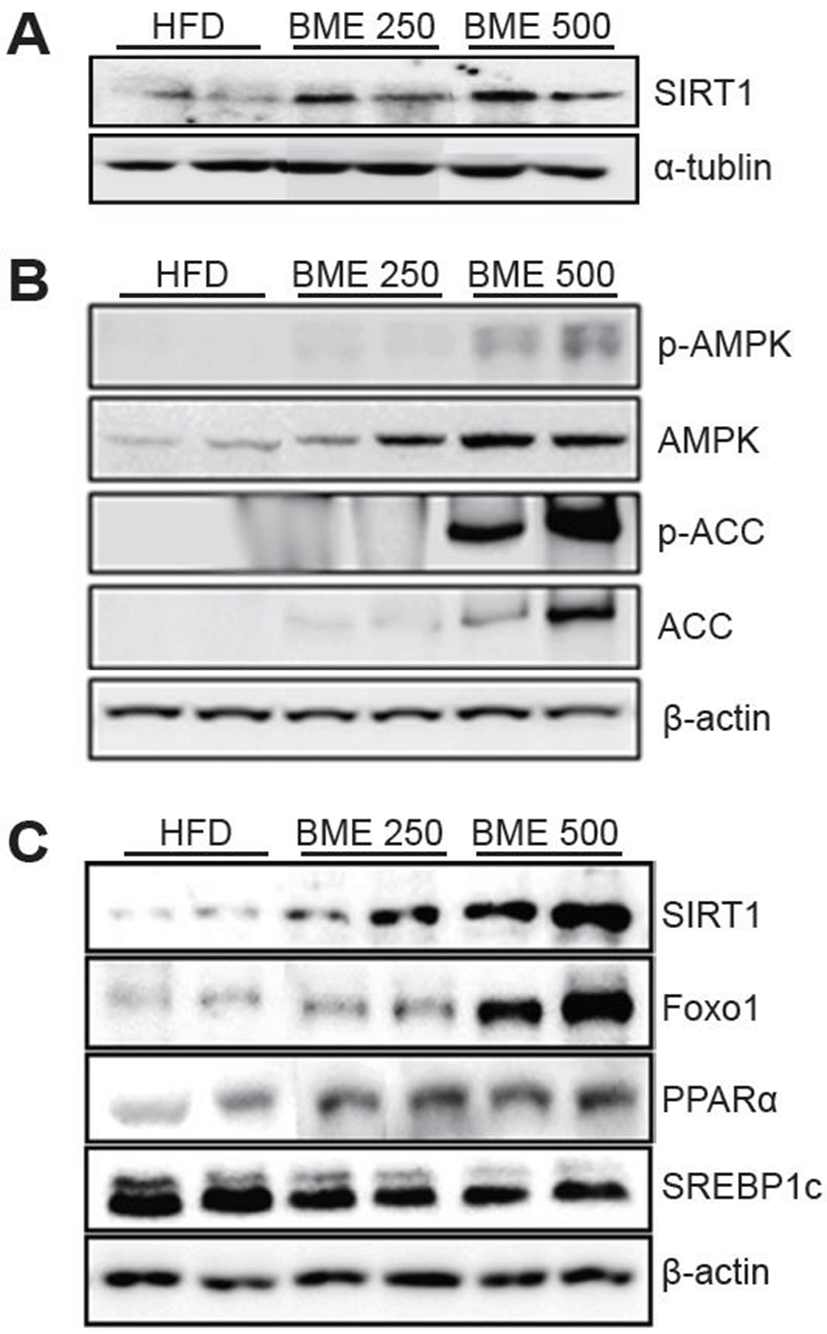
Discussion
In the present study, we demonstrated that the effect of bitter melon extract on the HFD-induced obesity and T2DM in C57BL/6 mice. Already numerous people use bitter melon for the prevention and primary therapeutics for the early T2DM like status. This study aimed to evaluate the precise effects of bitter melon on the lipid accumulation and development of T2DM. We used only premature bitter melon (before the formation of seed) to avoid the toxicity.
One of the hallmarks of the development of metabolic syndrome and T2DM is the elevation of ectopic lipid accumulation in non-subcutaneous adipose stores (increased central adiposity, muscle and liver lipid accumulation) (Lara-Castro & Garvey, 2008). BME inhibited visceral fat accumulation without affecting food intake. Therefore, it is suggested that an adequate administration of BME might effectively reduce hepatic steatosis. Actually, increased visceral adiposity is characterized as an important factor in the development of T2DM in human subjects (Bray et al., 2008; Ginsberg & MacCallum, 2009). Numerous studies have demonstrated the link between ectopic fat deposition and insulin resistance in persons with overt T2DM (Unger, 2003; Lara-Castro & Garvey, 2008). Momordica charantia is a food source that contains various bioactive compounds with health benefits (Ruda-Kucerova et al., 2015). Previous studies have shown that in mice fed HFD, freeze-dried Momordica charantia juice was found to possess the potential to reduce adiposity, and extracts from this plant may reduce visceral obesity (Chen et al., 2003; Alam et al., 2015; Mahmoud et al., 2017).
In present study, we investigated whether BME could improve HFD-induced obesity in C57BL/6 mice and explored the mechanisms. C57BL/6 mice were maintained on an ND or HFD for 12 weeks and then fed the ND, HFD, BME 250, or BME 500 for another 12 weeks. The weekly food intakes among the groups did not differ from each other. BME obviously reduced the weight of WAT and the liver in the HFD group, which was consistent with the results of changes in body weight. These results suggested that the BME-mediated decrease in body weight was attributable to a reduction in the weight of WAT and the liver independent of food intake.
It has been reported that hyperglycemia is the defined pathological feature of T2DM. Effective control of glucose levels is crucial for preventing diabetic complications and improving patients’ quality of life (Shafiee et al., 2012; Agrawal, 2016). Present results of IPGGT and IPITT suggested that BME may improve T2DM pathologies by alleviating hyperglycemia.
SIRT1 mediates metabolic benefits in various tissues. In the liver, SIRT1 supports gluconeogenesis via peroxisome proliferator-activated receptor (PPARα) and forkhead box O1 (FOXO1) (Rodgers et al., 2005) and inhibits fatty acid synthesis by targeting sterol regulatory element binding protein 1c (SREBP1c) for degradation (Walker et al., 2010). The down-signaling molecules of SIRT1, such as Foxo1 and PPARα were increased significantly and SREBP1c was decreased significant in the BME administrated animals. These findings showed that BME increased hepatic gluconeogenesis and fatty acid use by up-regulating a SIRT1 signaling pathway. As also, BME up-regulated the expression levels of p-AMPK and p-ACC. These data showed that on one hand, BME may up-regulate SIRT1 to induce gluconeogenesis; on the other hand, it may stimulate AMPK and ACC to promote fatty acid oxidation in the liver. Recent studies have shown that aqueous extract of Momordica charantia seeds regulated the insulin signaling pathway in muscles and adipose tissues primarily by targeting insulin receptors (IR) (Lo et al., 2013). Here, for the first time, we illustrated that BME reinforce HFD-induced gluconeogenesis and fatty acid use in the livers of mice by modulating SIRT1 signaling. SIRT1 is involved in protection against metabolic syndrome. However, SIRT1’s purpose in protecting against metabolic syndrome is known mostly through indirect evidence by using the chemical activators of SIRT1(Pfluger et al., 2008). Studies have demonstrated the beneficial effects of bitter melon extract on HFD-induced obesity, including the improvement of insulin sensitivity and hepatic steatosis by regulating the SIRT1 signaling pathway. Similarly, in this study, BME not only enhanced the expression levels of SIRT1 but also up-regulated Foxo1 and PPARα, as also down-regulated SREBP1c. In fact, the underlying mechanisms of hepatic metabolism in these animal models are different; it seems that BME may act on a particular pathway, depending on the cause of T2DM development. In this study, BME increased the phosphorylation levels of AMPK in the liver. Briefly, the stimulation of AMPK in the liver may be the consequence of a reduction in the AMPK/ACC pathway, not the SIRT1/ AMPK pathway.
In conclusion, BME not only attenuated HFD-induced hepatic steatosis but also ameliorated HFD-induced insulin resistance by improving insulin sensitivity. The reduced circulatory insulin inhibited expressions of lipogenic genes in the liver and attenuated hepatic steatosis.
In summary, BME greatly enhanced insulin sensitivity, reversed liver steatosis, reduced body-fat mass and increased the expression level of SIRT1 in mice with HFD-induced T2DM. This study suggested that the favorable effects of BME on increasing insulin sensitivity and attenuating hepatic steatosis may be attributed to the mediation of SIRT1 and AMPK-ACC signaling. Therefore, BME can be viewed as a potential natural resource for the development of new therapeutic alternatives in the treatment of obesity and T2DM.