INTRODUCTION
The early growth response (Egr) 1 is a member of the immediate-early gene family that includes Cys2–His2 class of zinc finger proteins. This gene encodes a 533 amino acid, 57 kDa protein containing three putative DNA- binding zinc finger elements (Sukhatme et al., 1987; McMahon et al., 1990). Early growth response protein 1 (Egr-1), a zinc finger transcription factor (TF) is, also known as KROX- 24, NGFI-A, ZIF-268, and TIS8 (Moratalla et al., 1992; Humblot et al., 1997; Topilko et al., 1997; Knapska & Kaczmarek, 2004). This protein activates transcription via a high-affinity regulatory element GCG(G/T)GGGCG in a zinc-dependent manner (Decker et al., 1998; Tremblay & Drouin, 1999).
Studies have shown Egr-1 mRNA during frog embryogenesis for the development of the bone and muscle, as well as several other mesodermal structures, including tooth mesenchyme and hair follicles (Mcmahon et al., 1990; Nentwich et al., 2009). In adult mice, the maximum expression levels of Egr-1 mRNA are restricted to the heart, brain, and lung, and the minimum expression levels, to the spleen and kidney (Milbrandt, 1987; Christy et al., 1988; Lemaire et al., 1988; Langmann, 2007). In zebra fish, several tissues express Egr-1, including the hypothalamus, pharynx, retina, and optic vesicle during embryogenesis (Close et al., 2002; Langmann, 2007).
Initially, Egr-1 was considered to be an immediate early gene that rapidly responded to different growth stimuli and involved in cell proliferation, differentiation, and development, because it was induced in quiescent fibroblasts exposed to serum growth factors (Nguyen et al., 1993; Svaren et al., 1996). Egr-1 binds to GC-rich, cis-acting promoter elements (Khachigian et al., 1997; Fernandez-Alvarez et al., 2008), and controls the expression of a wide variety of genes relevant to pathogenesis (Hasan et al., 2003; Blaschke et al., 2004), including growth factors, cytokines, receptors, and proteases, many of which are involved in angiogenesis (Lucerna et al., 2003). For example, the expression of Egr-1 has been shown to be activated by acidic fibroblast growth factor (aFGF), basic fibroblast growth factor (bFGF), vascular endothelial growth factor (VEGF), and shear stress (Silverman & Collins, 1999; Urakawa et al., 2006).
Egr-1 mediates by angiogenesis through the activation of the vascular endothelial growth factor (VEGF) gene (Mechtcheriakova et al., 1999; Liu et al., 2000). Thus, angiogenesis that occurs during the development stage leads to the formation of the gill vascular system. This is essential for gas exchange and respiratory metabolism during fish embryogenesis. The gill vascular system of teleosts, elasmobranchs, and dipnoans has been researched extensively over the last a few years (Kimmel et al., 1995). However, the role of Egr-1 expression in gill vascular system formation during embryogenesis is still unclear.
Here, we investigated the role of the growth factor inducible Egr-1 in gill vascular formation during embryogenesis of olive flounders. We also assessed the accompanied changes in the expression patterns of Egr-1 from fertilization to post-hatching period. Additionally, we examined the expression patterns of Egr-1 in several tissues of the olive flounder.
MATERIALS AND METHODS
All experimental fish were raised at Genetics and Breeding Research Center, National Fisheries Research and Development Institute (NFRDI) and maintained in 10 tons flow through tank at 20±1°C under a natural photoperiod. Different stages of embryo (0.92±0.02 mm), larvae (2.49± 0.34 mm) and juvenile (3.5±0.45 mm) development were described from fish kept at 20°C in the tank. Several tissues of 2 years (38 cm±SEM) old were used to in olive flounder. The samples of 10 fish were randomly collected and frozen in 70°C deep freezer until isolation of total RNA. Pooled several tissues were homogenized for 20 sec with Trizol reagent (Invitrogen, Carlsbad, CA, USA).
Larvae were examined as previously described, fixed at room temperature, in 2.5% glutaraldehyde (Polysciences, Inc., Warrington, PA) in 0.1 M sodium cacodylate–HCl buffer, pH 7.3, for 10 min, and post fixed in 1% paraformaldehyde (Sigma-Aldrich, CA, USA) in the same buffer, for 20 min with 1% uranyl acetate for 1 h and ethanol dehydration. The samples were examined under a stereo microscope (ZEISS CL1500 ECO Jena, Germany) imaging system at ×400 magnification of development was determined. The 5 days after hatching stage of gill vessels system development were illustrated using a Power point (Microsoft, WAS., USA)
Total RNA was extracted using the Trizol Reagent (Invitrogen, Carlsbad, CA, USA) according to the manufacturer's protocol. DNase-I (Sigma-Aldrich, CA, USA) was treated for removing genomic DNA contamination from RNA for 30 min at 37°C. The RNA samples were extracted with phenol/chloroform to inactivate the DNase I. RNA concentration was measured with spectrophotometer (Gene-Quant, Pharmacia Biotech), quality of RNA was checked by gel electrophoresis (1% agarose gel) and stored at –80°C until further use. RNA (100 ng) from each sample was transcribed to cDNA using RT-&GO Mastermix (Qbiogene, Inc., Virgi., USA). The amplification was performed with AmpliTag Gold DNA Polymerase (Applied Biosystems., CA, USA) and Veriti Thermal Cycler (Applied Biosystems., CA, USA) using the following parameters: denaturation at 95°C for 10 minutes and 35 cycles of reactions of denaturation at 98°C for 10 s, annealing at 58°C for 30 s, and elongation at 72°C for 45 s. An aliquot of each PCR product was subjected to 1.5% (w/v) agarose gel electrophoresis and visualized by staining with Red Safe (Intron, Seoul, Korea). A search of the National Center for Biotechnology Information (NCBI) data base revealed a partial sequence similar to that of Egr-1 (KC442103.1). The 5' forward and 3' reverse-complement PCR primers for amplification of each gene were as follows: Egr-1 (forward primer: 5'-CCTGCCTA CCCAAATAGCAA-3' and reverse primer 5'-GTGGATGCGAATATGTCGTG- 3'), β-Actin (forward primer: 5'-TGATGAAGCCCAGA GCAAGA-3' and reverse primer 5'-CTCCATGTCATCC CAGTTGG-3'). Relative amount of each messenger RNA was quantified by dividing by density of housekeeping gene (Gene bank, HQ 386788.1).
To evaluate arrestin mRNA levels, these primers were specifically designed to detect and quantify cDNA sequences without detecting genomic DNA. The Fast-Start DNA Master SYBR Green I (Roche Ltd., SWISS) was used as fluorescent reporter dye to detect amplification products in 7500 Fast Real Time PCR System (Applied Biosystems Inc. Carlsbad, CA, USA) using the following cycling conditions: denaturation at 95°C for 10 min and 40 cycles of reactions of denaturation at 95°C for 10 s, annealing at 58°C for 30 s, and elongation at 72°C for 30 s. Each sample was tested in triplicate to ensure statistical significance. The PCR efficiency (E%) of gene was derived from perforin (96.2%) and b-Actin (95.4%) respectively. The relative quantification of arrestin gene expression was performed using the comparative Ct method. The Ct value is defined as the point where a statistically significant increase in the fluorescence has occurred. The number of PCR cycles (Ct) required for the ROX intensities to exceed a threshold just above background was calculated for the test and reference reactions. In all experiments, β-Actin was used as the endogenous control. Results were analyzed in a relative quantitation study with the vehicle treated.
Data were analyzed using Sigma plot for Windows (Jandel Scientific, San Rafael, CA, USA). For unpaired matched comparative analysis of multiple groups, an analysis of variance (ANOVA) was performed. Data that did not meet normality assumptions were subjected to one way ANOVA on ranks, and then pair wise comparisons were made using the Student-Newman-Keuls (SNK) method.
RESULTS
Microscopic analysis of gill vessels formation was performed at the end of the embryonic stage and at the beginning of larval development of the flatfish. The period of embryogenesis in the olive flounder is about 5 days after hatching (DAH) at 20°C. We first observed the lateral side of the live olive flounder larvae 5 DAH by using a dissecting stereomicroscope. At this stage, the eye, heart, gill filament, auditory capsule, hyoid arch vessels, and gill arch vessels were visible, which are all generated from the back of the eyes (Fig. 1A). At this stage, the blood vessels of the gill, hyoid, and gill arches of the calculate system function as provisory respiration organs (Fig. 1A). To determine the origin of the gill vessels system during embryonic development in the olive flounder, larvae 3.5 mm in length were observed. Within 5 DAH, most of the gill filaments already contained capillary vessels, and these were present in almost all gill filaments by 7 DAH. Just after hatching, rudiments of the gill filaments were formed on the gill arches. Within 5 DAH, the gill filaments had begun to branch and contained gill capillaries. A gill slit was formed posterior to the hyoid arch and between each branchial arch, forming five slits in all. The rudiments of the gill filaments develop late in the hatching stage as buds along the posterior walls of the branchial arches facing the slits. The gill vessels system in the 5-DAH larvae consisted of gill arch vessels, hyoid arch vessels, and gill filaments. Figure 1A shows the gill vessels under the auditory capsule at 5 DAH and the gill filaments budding laterally to connect with the gill arch vessels, which function as the circulation system.
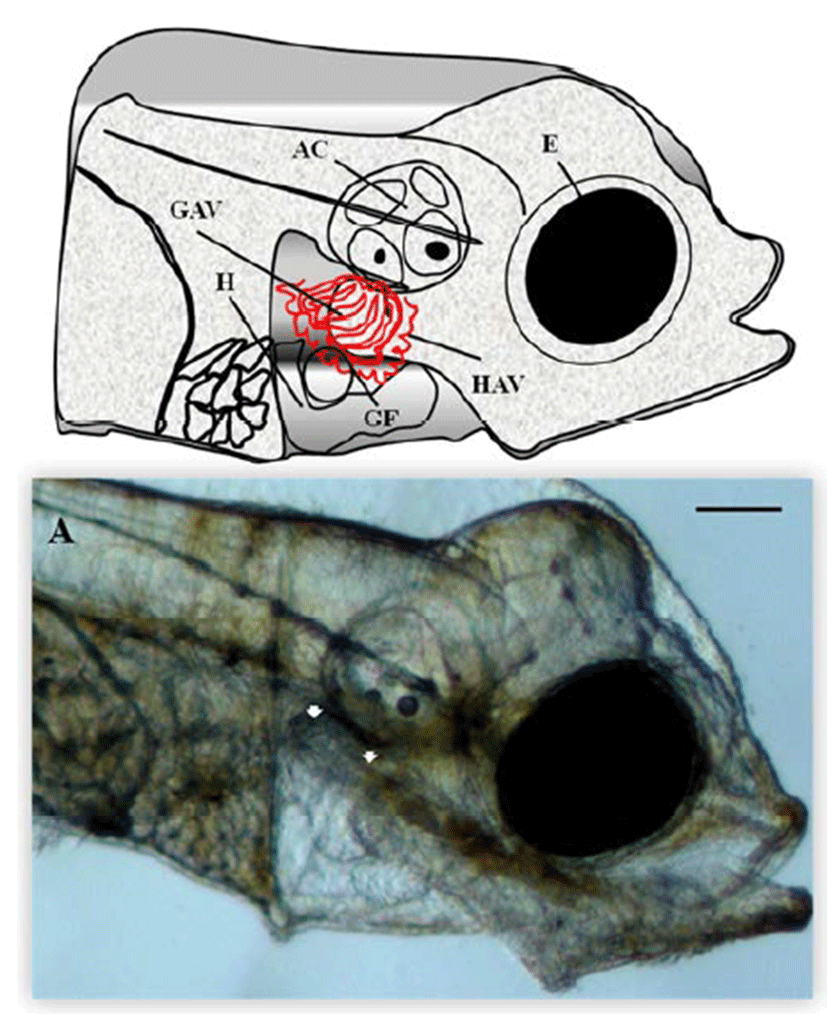
Egr-1 expression was measured during olive flounder embryogenesis and in several tissues by using traditional reverse transcription-polymerase chain reaction (RT-PCR). Egr-1 gene induction was not observed during the first stages of development from the fertilization stage to the gastrula stage. Egr-1 expression was first detected at the beginning of the neurula stage, and then increased continually until the larval period, and strong expression was observed at 5 DAH (Fig. 2A). High egr-1 expression was observed in the spleen and gills, and lower expression levels were observed in the fins, eyes, and kidneys (Fig 3A). Egr-1 expression in olive flounder during embryogenesis and in various organs from adults was also examined using realtime time PCR (Fig. 2B, 3B). Similar egr-1 expression levels were observed from the neurula period to 5 DAH. In adults, egr-1 mRNA was expressed at similar levels in the spleen and gills, but lower expression was observed in the fins, eyes, and kidneys (Fig. 2B, 3B). These results show that egr-1 is more strongly expressed during development of the gill vessels system, which suggests that it plays a role in gill vessels formation during embryogenesis.
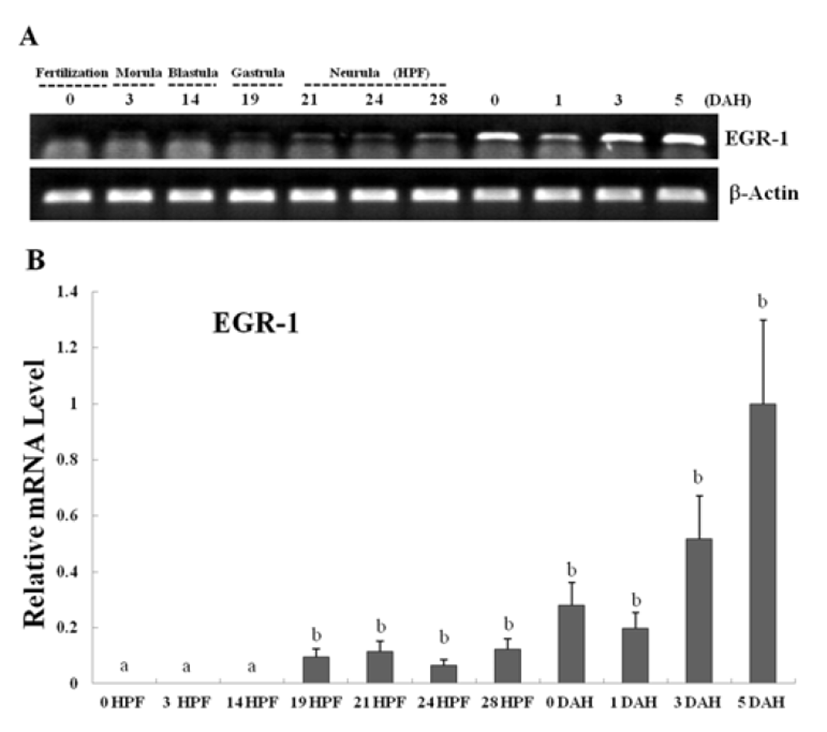
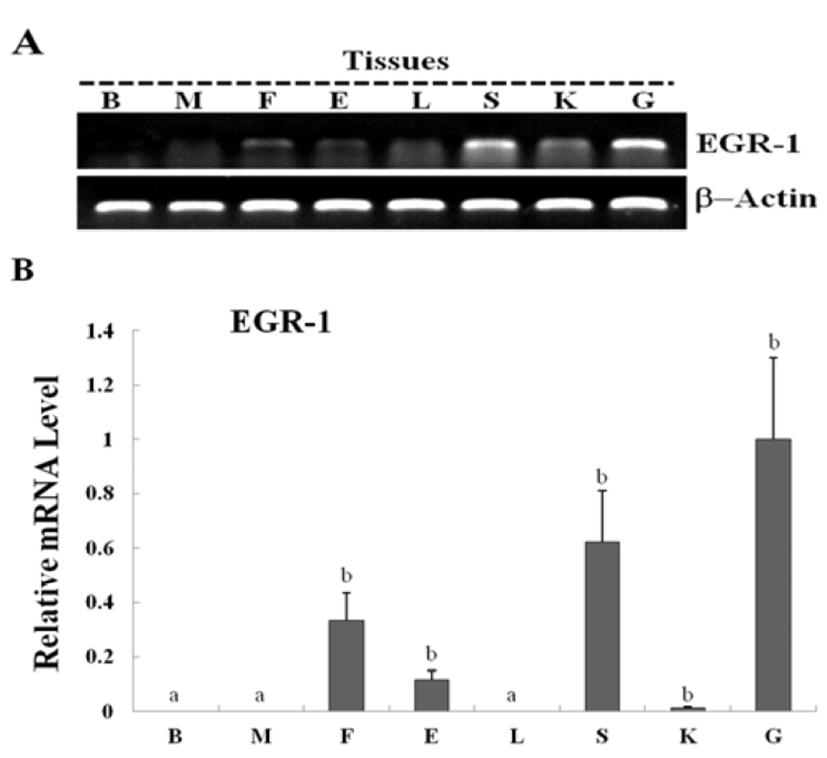
DISCUSSION
The change from embryogenesis to the larval stage of growth in fish is accompanied by regulated substitution of the provisory respiratory organs with the definitive organs (Kimmel et al., 1995). Previous studies have established that the gill vessels system is fully formed and egr-1 expression is detected within days after hatching in zebra fish (Oxtoby & Jowett, 1993; Close et al., 2002). However, the formation of the gill vessels system in relation to expression of the egr-1 gene has not been described previously. Guided by the position of the mature gill vessels system, we examined the cellular structures medial to the blood cartilages of the gill vessels system at various time points during development to define the stages of vascular system formation. At 5 DAH, a gill filament was observed lateral to the head (Fig. 1A). The development of the formation of the initial vascular system expressed in embryogenesis is also preserved at later developmental stages. For example, stickleback larvae have gills with various filaments that already begin to branch and contain capillary vessels by the beginning of the transition to the opened mouth stage (Swarup, 1958; Shadrin & Ozerniuk, 2002). By contrast, in the zebrafish, at the initial stages of metamorphosis (about 18 DAH), the larvae already have an almost complete definitive gill system. In olive flounder, formation of the gill vessels system is an essential component of the development of respiratory metabolism and is dependent on the expression of VEGF during embryogenesis (Scehnet et al., 2007; Trindade et al., 2008). VEGF/ VEGFR signaling pathways are essential for vascular and hematopoietic cell development (Giles, 2001). Gain-offunction studies have shown that VEGF expression in endothelial cells is mediated by Egr-1 (Liu et al., 2000). Our data showed that Egr-1 mRNA was expressed and detectable until 5 DAH in olive flounder (Fig. 2A, B). Systemically administered VEGF led to increased Egr-1 protein levels in the micro-vascular endothelium of the liver and heart, and administration of EGF resulted in Egr- 1 induction in the micro-vascular endothelial cells of the skeletal muscle (Gerritsen, 1987; Silverman & Collins, 1999). Recently, Egr-1 protein was shown to be expressed in a variety of cells in adult mice, including hepatocytes, neuronal cells, cardiomyocytes, and endothelial and vascular smooth muscle cells (Cohen et al., 1996). Inducible Egr-1 expression may coordinate the expression of multiple target genes involved in cell movement and replication in blood vessel walls (Ayadi et al., 2001). The vascular system has a mesodermal origin, and there is evidence that Egr-1 plays an important role in vascular biology (Lejard et al.; Mechtcheriakova et al., 2001). Similar to previous reports, we also found that egr-1 is expressed at relatively high levels in the gill vessels system and lymphoid organs in the tissues of adult flounder (Fig. 3A, B). Gill vessels system formation was observed in the larval stages, and the Egr-1 gene was significantly expressed in these stages, suggesting that Egr-1 may play a crucial role in the larval respiration vessels system.
In summary, expression analysis of Egr-1 during gill vessels formation might provide information that could be useful in understanding the initial respiration responses and growth of olive flounder larvae. Further functional studies of this gene will help to elucidate the molecular regulatory mechanisms and the stress response involved in gill vessels formation in olive flounder.