INTRODUCTION
Ocean acidification, caused by increasing atmospheric concentrations of CO2, is one of the most critical anthropogenic threats to marine life (Kim et al., 2015). The uptake of anthropogenic carbon dioxide (CO2) by oceans has induced fundamental changes in seawater chemistry, which could have dramatic effects on ecosystems in the upper ocean (Gattuso and Hansson, 2011). Atmospheric CO2 diffuses passively at the ocean surface, forming carbonic acid, which dissociates into hydrogen and bicarbonate, and reduces pH (Fabry et al., 2008). In general, marine pH normally ranges from 7.8 to 8.2 (Knutzen et al., 1981) but increased dissolution of atmospheric CO2 has led to decreases in pH. The pH of water is one of the main environmental factors exerting a selective pressure on marine and freshwater organisms, interfering with their ability to maintain homeostasis. As such, ocean acidification has become a central scientific issue in recent marine ecosystem research (Feely et al., 2009).
Osmolality and pH can have both direct and indirect effects on aquatic organisms. Cells of organisms can survive by actively preventing the absorption and release of minerals and by releasing some of the incoming minerals and reabsorption of drained water; this involves the expenditure of energy in the form of adenosine triphosphate (ATP). Alternatively, when the concentration of inorganic salts in the immediate environment is lower than that in body fluids, inorganic salts are released from the cell passively. Since the concentration of inorganic compounds in body fluids is not equal to their concentration in the surrounding water, energy is expended (in the form of ATP) to maintain homeostasis. Therefore, aquatic organisms are always regulating osmolality, either actively or passively.
Many studies have investigated the effects of pH variability on organisms in freshwater systems, where the environment can be subject to dramatic fluctuations. Numerous taxa, including trout, cichlids, and frogs, have shown osmotic and ionic imbalances as a result of acidic environments (Henriksen et al., 1984; Shu et al., 2015). Under projected climatic conditions, marine organisms may be subject to similar physiological pressures. Studies have shown that rainbow trout (Oncorhynchus mykiss), Atlantic salmon (Salmo salar), moor frog (Rana arvalis), and cichlid fish species are vulnerable to low pH. In rainbow trout, ionic loss caused plasma osmotic pressure to fall precipitously at pH 4.2 (Henriksen et al., 1984). In Atlantic salmon, rapid changes in pH and calcium and aluminum speciation may be a major reason for fish mortality (Henriksen et al., 1984). A study on moor frogs (Shu et al., 2015) showed that different ion channels and pumps were employed for embryonic survival under acid stress. In two cichlid fish species of the Amazon, discus fish were more tolerant to low pH than angelfish (Pterophyllum), as seen by the lesser effects of exposure to pH 3.6 on unidirectional Na+ uptake and efflux rates and net Na+ and Cl− losses in the former species (Duarte et al., 2013). Although reductions in pH may negatively affect the survival, growth, reproduction, and physiological performance of marine organisms, variations in carbonate chemistry have detrimental effects on marine calcifiers, as the production of calcareous skeletal structures is hindered (Fabry et al., 2008). A study of orange clownfish (Amphiprion percula), revealed a decline in olfactory sensing capability under high CO2 conditions (Kim et al., 2015). Dixson et al. (2010) found that fish raised at higher CO2 levels could not easily escape from predators because they were not able to smell predators efficiently. Usually, fish larvae and eggs are more sensitive to pH changes than adult fish (Brown and Lynam, 1981) and the effects of ocean acidification are, therefore, more likely to be detected during these early life stages (Checkley et al., 2009). Previous studies have indicated that pH changes can induce serious ion deficiencies in seawater. While there are many studies of the effects of water acidification on freshwater fish species and amphibians, such studies are scarce in marine fishes.
In aquaculture facilities, water can become acidified due to nitrate and CO2 accumulation. Coastal areas and fish farms may also become acidified by nitrates from feed and drugs. The olive flounder (Paralichthys olivaceus) is a commercially important farmed fish species in China, Japan, and Korea with more than 43,000 tons cultured annually in Korean hatcheries, particularly on Jeju Island. In this study, we investigated the effects of pH on the spawning and hatching of P. olivaceus. We hypothesized that the fertilized eggs and larvae of P. olivaceus would be adversely affected under low pH conditions, particularly in terms of decreased osmotic pressure leading to high mortality and damage to fertilized eggs and larval skeletons. The innate immune response is fundamental defense response of vertebrates and invertebrates. Especially, the innate immune response important for larvae that lack of resistance to infectious in the early stages. Caspase, galectin-1, and HSP 70 are associated with immune function related to apoptosis, external mucous, and protection of impaired proteins, respectively. We assessed these hypotheses by examining ontogenic changes, osmolality, and expression of several immunity-related genes (caspase, galectin-1, and heat shock protein 70) in P. olivaceus eggs and larvae exposed to different pH levels.
MATERIALS AND METHODS
Three different pH levels were used: 4.0, 6.0, and 8.0 (natural seawater, as a control) (salinity 35 ppt). To attain each of these pH levels, approximately 10 L of natural seawater was passed through a filter flask and pH levels were adjusted using HCl or NaCl. During the experiment, pH and salinity were calculated every 5 h using handheld pH meters (YSI 5909; YSI, Yellow Springs, OH, USA), which were calibrated daily, and levels were adjusted used HCl or NaCl. Temperature and light were maintained at 19±0.5°C and 12 h light/12 h dark, respectively.
Unfertilized eggs and semen of P. olivaceus were obtained from the National Institute of Fisheries Science (NIFS) in Wimi-Ri, Jeju, Korea. Adult mature P. olivaceus three males and four females were anesthetized in 100 ppm of 2-phenoxyethanol. Semen and unfertilized eggs were collected by manual abdominal stripping at 20°C water temperature and 31.25±0.43 salinity and were stored at 4°C. To achieve artificial fertilization, the stripped eggs and semen were mixed dry and then the coelomic fluid was removed by washing the fertilized eggs with natural seawater three times in a bucket.
Two-liter beakers (three beakers per treatment for a total of nine) were seeded with 500 fertilized eggs. The overall survival rate in each was calculated, and embryonic development (over 38 h) was monitored from zygotic cleavage to near-hatch readiness using an optical electron microscope (DE/LMD07000; Leica Microsystems, Wetzlar, Germany). Ontogenic stages were defined, for all pH groups, as two-cell, four-cell, thirty-two-cell, morula, blastula, embryonic, heart differentiation, and pre-hatch stages. Survival rates of larvae were also calculated from the day of hatching for five days.
Osmolality was calculated for all pH treatments at the two-cell, blastula, embryonic, Kupffer’s vesicle formation, and pre-hatch stages, and in post-hatch larvae for five days. Measurements were carried out using 20 fertilized eggs and 50 live larvae washed with Kimtech wipers in each beaker, washed three times with distilled water (DW) for each pH group, then homogenized and centrifuged at 15,000 ×g at 4°C for 10 min. The osmolality of the supernatant was calculated from three measurements using a vapor pressure osmometer (Micro Osmometer 3300; Advanced Instruments, Norwood, MA, USA).
Transcriptional gene expression levels were calculated from 10 fertilized eggs at the two-cell, blastula, embryonic, Kupffer’s vesicle formation, and pre-hatch stages, and five larvae were assessed on day 5. Total RNA was extracted using TRIzol (Life Technologies, Carlsbad, CA, USA) according to the manufacturer’s instructions. One microgram of extracted RNA was treated with DNase I (Fermentas, Hanover, MD, USA) and used to synthesize cDNA using a Transcriptor First Strand cDNA Synthesis Kit (TaKaRa Bio, Kusatsu, Japan). Quantitative real time PCR was conducted using the TOPrealTM qPCR 2× PreMIX (SYBR Green with ROX, enzynomics). The primers for qPCR analysis of caspase, galectin-1, and heat shock protein-70 (HSP 70) genes in P. olivaceus were designed using the Primer 3 program (Ver. 0.4.0, http://frodo.wi.mit.edu/primer3; Table 1). PCR reactions were carried out at 95°C for 10 min, followed by 45 cycles at 95°C for 10 s, 60°C for 15 s, and 72°C for 30 s, plus a final stage at 72°C for 30 s.
RESULTS
There were no differences in eggs among treatments from the beginning of hatching to 30 h (embryonic stage). We found that each head part varied in size among treatments at the heart differentiation stage and 1–5 days post-hatching (Fig. 1). After 38 h, fertilized eggs showed differences in head size. We observed larger head sizes in the pH 6.0 treatment than pH 4.0 and 8.0 levels. There was no evidence of differences in morphology across zygotes reared in different pH environments until the embryonic stage. However, zygotes at pH 4.0 developed considerably more slowly than those at pH 6.0 and 8.0 levels (Fig. 2). After 38 h, all embryos in the pH 4.0 treatment either sank or failed to hatch, yielding a mortality rate of 100%; mortality for pH 6.0 and 8.0 levels ranged from 66.5% to 69.4% (Table 2). And no significant differences in morphology were seen from the two-cell stage until the blastula stage.
pH section | Number of demersal eggs | Percentage (%) |
---|---|---|
pH 4.0 | 500±0.00 | 100 |
pH 6.0 | 167.67±30 | 33.5 |
pH 8.0 | 153.00±46 | 30.6 |
Hatching occurred more slowly at pH 4.0 and 6.0 (38 h vs. 30 h; Fig. 1) and did not occur at all at pH 4.0 (Table 2). The mortality rates of larvae at pH 6.0 and 8.0 were 8.43% and 6.44%, respectively (Table 3).
pH section | 1 day | 2 days | 3 days | Number of deaths | Death rate (%) |
---|---|---|---|---|---|
pH 4.0 | 0 | 0 | 0 | 0 | 0 |
pH 6.0 | 4.00±2 | 8.33±9 | 15.67±7 | 28.00±14 | 8.43 |
pH 8.0 | 7.33±4 | 7.67±3 | 7.33±6 | 22.33±0.58 | 6.44 |
Expression profiles showed that all three genes were expressed at all pH levels (Fig. 3). Caspase and galectin-1 were expressed from the blastula to pre-hatch stages, with the exception of the two-cell stage. Caspase was much more strongly expressed at pH 4.0 and 6.0 than at pH 8.0. This expression increased over time in developing embryos at pH 6.0. Galectin-1 was continuously expressed at the pre-hatch stage, but expression decreased with time. HSP 70 was also expressed at all pH levels after the two-cell stage. In particular, HSP 70 expression was similar from the blastula stage until the pre-hatch stage. Post-hatch, beta actin was expressed at all pH levels (Fig. 4). HSP 70 was also steadily expressed at all pH levels over the five days. However, caspase and galectin-1 were not expressed at pH 8.0 post hatch.
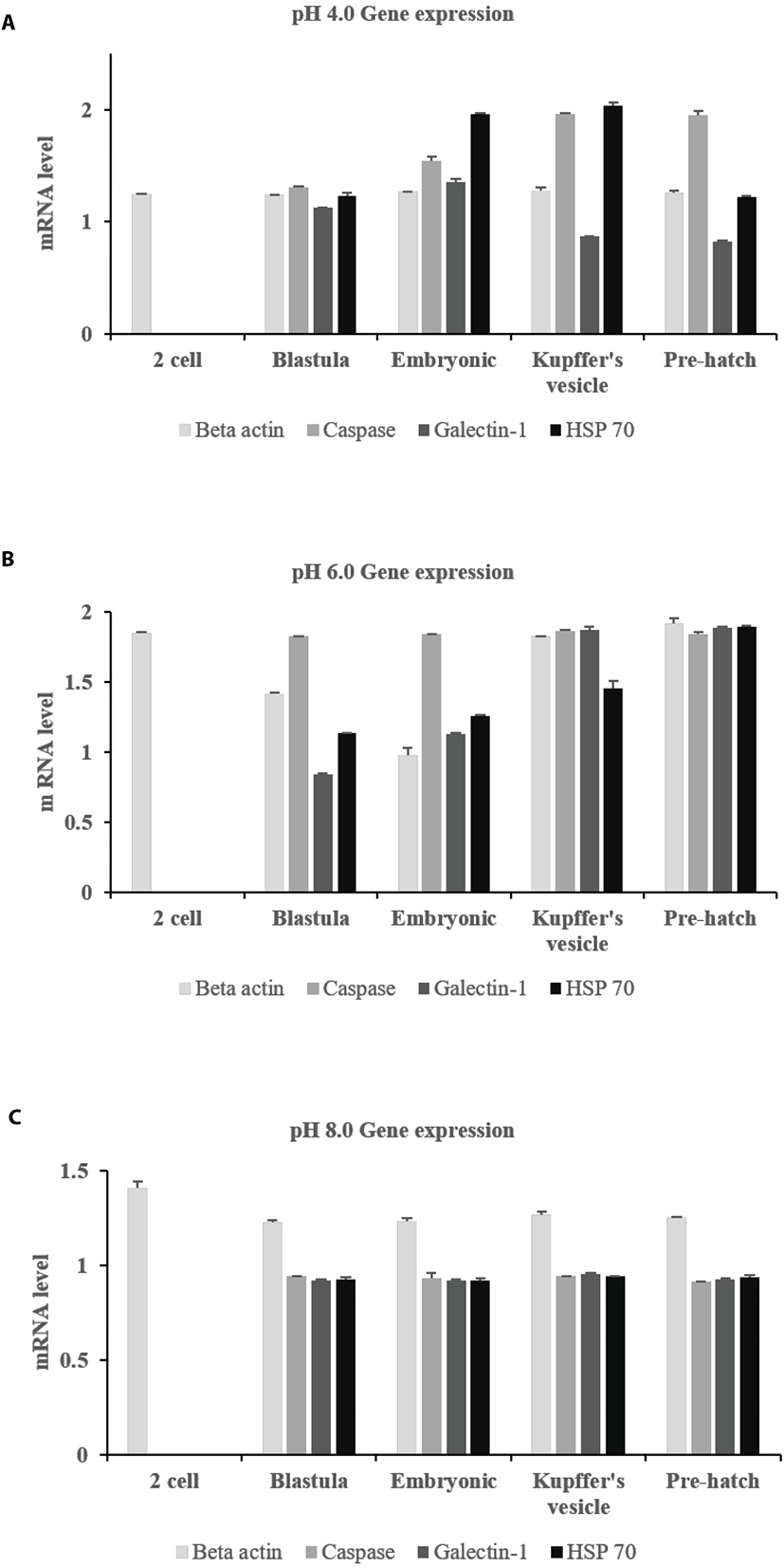
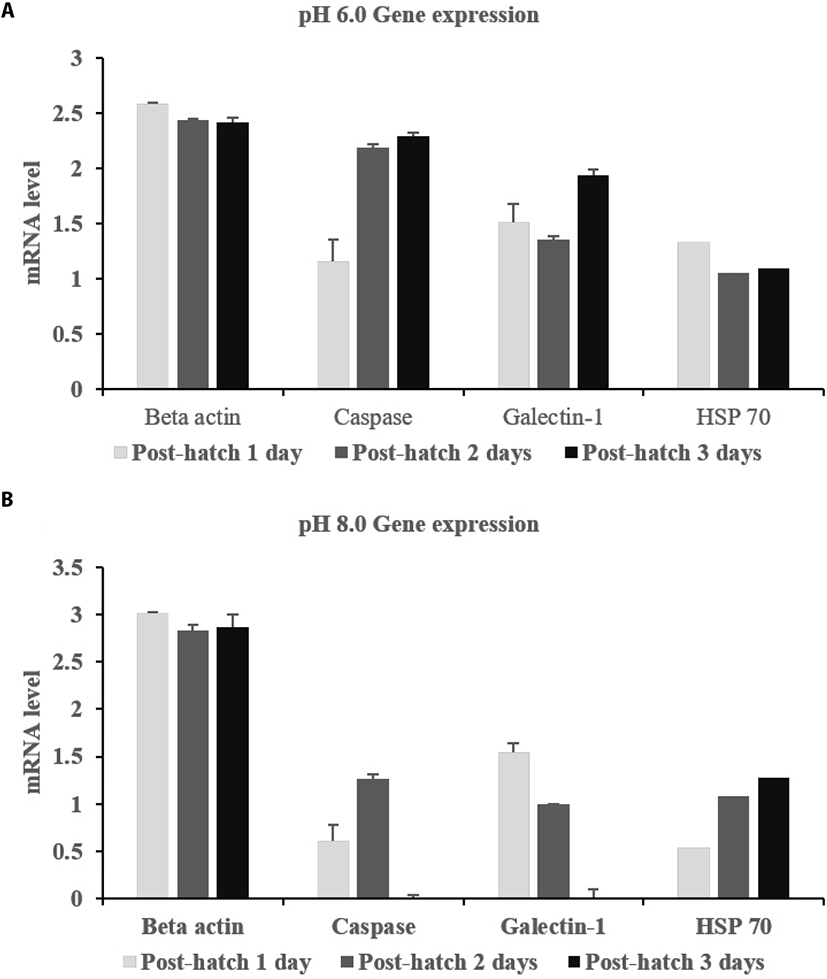
The osmolality of fertilized eggs was below 200 mmol/kg, which was maintained until after the embryonic stage in all pH groups (Fig. 5A). At the pre-hatch stage, osmolality was not significant but at the Kupffer’s vesicle formation stage it was significant in all pH groups. Osmolality increased from the two-cell stage until the blastula stage but was unchanged between the embryonic and prehatch stages. At later stages, osmolality increased considerably. Two days after hatching, osmolality was significantly higher at pH 8.0 (natural seawater), but there was no significant difference in osmolality between the other pH groups (Fig. 5B).
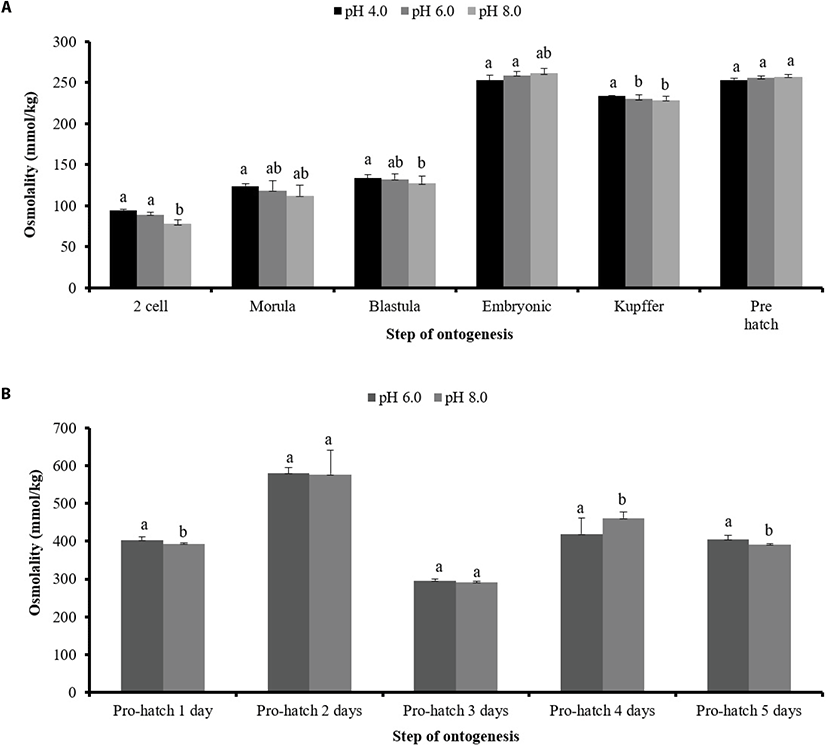
DISCUSSION
Our investigation of morphology, hatch rate, osmolality and gene expression at different pH levels revealed that an acidic environment harms the development of the fertilized eggs and larvae of P. olivaceus. At pH 4.0, eggs showed delayed differentiation and eventually sank to the bottom. Generally, the hatching enzyme (chorionase or envelysin) is secreted by the integument of the snout (Bell et al., 1969) to digest much of the zona radiata (Peterson et al., 1980). Many studies have reported hatch failure or slow growth of fertilized eggs at low pH. The hatching delay described above was observed at low pH and is similar to the results of previous studies (Daye and Garside, 1980). In contrast, Trojnar reported that hatching of brook trout eggs reared at low pH was more rapid than at higher pH values (Trojnar et al., 1977). In Atlantic salmon, hatching of eggs in acidic water was delayed by six days at pH 4.5–5.0, and did not occur at all at pH 4.0 to 4.2 (Peterson et al., 1980). We hypothesize that low pH can affect hatching enzyme secretion. We also found that both acidification and alkalinization of water affected spawning and led to increased egg mortality at levels comparable to those found in other marine and freshwater species, as demonstrated by similar mortality rates seen at pH 6.0 and 8.0 (Henriksen et al., 1984). Generally, the osmolalities of seawater and freshwater are in contrast with each other. Osmolality at pH 4.0 was not significantly different from that at pH 6.0 and 8.0, except at the Kupffer’s stage. This is assumed to be due to the formation of chloride cells at this stage. Acute exposure to acidified water results in a fall in the osmotic value of the blood: the lower the pH of water. In this study, an electron micrograph of a chloride cell exposed to acidified water showed that the tubes of the tubular system were swollen, a sign of early aging of the cell, probably caused by the low pH. At two days post hatching, osmolality had increased for all pH treatments and was not significantly different between pH 6.0 and 8.0. With sublethal water acidification, the osmotic value often remains very low for weeks, as has been observed with many species of trout and goldfish (Bonga and Dederen, 1986).
Numerous studies have investigated fish behavior in relation to a wide variety of environmental factors, but information on the effects of pH on fish behavior is sparse (Scott et al., 2005). We observed that the head size of developing embryos was affected by pH, with embryos developing at pH 6.0 having larger head size, however, this effect was not evident at pH 4.0. Survival rates in natural seawater were higher (Table 2 and Fig. 1) at both the fertilized egg and larval stages, and so differences in growth and ontogeny were assumed to be related to low pH. At 38 h, eggs at pH 6.0 and 8.0 hatched (Fig. 2); all eggs at pH 4.0 sank out of the water column. Fluctuating pH, Ca ion, and Al levels in water can be lethal to fish (Henriksen, 1984). Carline et al. (1992) reported that radio-tracked brook trout (Salvelinus fontinalis) migrated downstream in response to acid runoff, though it is unclear whether this response was due to elevated aluminum or low pH (Carline et al., 1992). We assume that mortality in P. olivaceus eggs was induced by low pH and related to a lower Ca ion concentration.
Generally, the exposure of the skin to acid makes it more susceptible to disease (McDonald, 1983; McWilliams and Potts, 1978). Of the genes we investigated, we found that the expression of caspase, which is associated with apoptosis, was highest at pH 4.0 and 6.0 (Fig. 3 and 4). Its expression increased with time, suggesting that it may hamper embryonic development. Caspase was also highly expressed at pH 8.0. Hence, we assume that it is more related to growth at low than at high pH. Galcetin-1 is related to mucus production in the skin and was more highly expressed at pH 6.0 than at pH 4.0. Its expression was more uniform in pH 6.0 and 8.0 at the pre-hatch stage. At this stage, thinning of membranes by increased ion exchange is assumed to occur. Galectin-1 is primarily involved in skin barrier function and is expressed in digestive tissues against pathogen infection. The digestive tract developmental period is important for protection against pathogen invasion and galectin-1 can aid in this aspect as well (Jang et al., 2013). Galectin-1 expression levels decreased with time at most pH levels, but it was continuously expressed at pH 6.0. Meanwhile, HSP 70 is known to be an important part of the cell machinery for protein folding, and helps to protect cells from stress. This gene was expressed at all pH levels at both the fertilized egg and larval stages. Its expression decreased with time at pH 6.0, but increased with time at the larval stage at pH 8.0 (Fig. 4). This suggests a possible deleterious effect of low pH on the stable expression of the galectin-1 and HSP 70 genes.
The observed effects on osmolality are unusual in marine fishes, which may offer insight into the evolution of osmolality and ionoregulation (Shartau et al., 2017). Generally, fish osmolality is approximately 300 mmol/kg and is controlled by chloride cells and the yolk sac in fertilized eggs and gills through Na+/K+ ATPase. Here, osmolality was below 200 mmol/kg until the embryonic stage and >200 afterward, at all pH levels (until the pre-hatch stage). Osmolality in acid-stressed rainbow trout was under 300 mmol/kg (Lee et al., 1983), which is consistent with our results. Therefore, we speculate that osmolality is related to the chloride cells and yolk sacs of fertilized eggs. Ion homeostasis in freshwater fish is achieved using specialized branchial transport mechanisms for active ion uptake from environments that are hypotonic to the blood, plus a tight gill epithelium to minimize ionic diffusive losses (Evans., 2011; Hwang et al., 2011). Generally, gill epithelia are considered to be the major sites of ion regulation in both freshwater and marine teleosts, with up to 90% of all acid-base transport achieved in these tissues (Heisler, 1986). Common to these mechanisms for Na+ uptake is Na+/K+ ATPase (NKA) in the basolateral membrane of gill ionocytes, which exports Na+ into the blood and contributes to the electrochemical gradient that drives Na+ uptake across the apical membrane (Hwang et al., 2011). The osmolality of fish at the pre-hatch stage was not notable, however, two days after hatching, at all pH levels, osmolality was higher than at any other point in time. The reasons for this are unclear and require further investigation into ion exchange. Low pH-induced fish or egg mortality may result from ion transport defects in Na+/K+ ATPase, which cause reduced osmotic pressure (Bonga and Dederen, 1986). Furthermore, low pH stimulates appetite and dietary intake of larvae because they must actively excrete excess ions to maintain their osmotic balance (Kim et al., 2015). Marine fish constantly counteract osmotic water loss to their hyperosmotic environment by drinking, i.e., absorbing salt and water across the gastrointestinal tract, and actively secreting the surplus salt load at the gills (Michael et al., 2016). Chloride ions enter via the Na+ gradient provided by Na+/K+ ATPase and are secreted at the apical membrane through CFTR-type (cystic fibrosis transmembrane conductance regulator) ion channels. Na+ ions flow passively through paracellular tight junction pathways (Evans et al., 2005; Hwang et al., 2011; Michael et al., 2016). Osmoregulators maintain a relatively constant internal osmotic pressure over a range of environmental salinities (Foshtomi et al., 2007). It is assumed that salinity and pH are correlated. A study of moor frogs revealed that ion channels and pumps are responsible for ion flux in cells, and are key mechanisms mediating cellular function. The resulting ion flux can mediate adaptive divergence of natural populations at early life stages in the face of environmental stress (Shu et al., 2015). Notably, two days after hatching, osmolality was significantly higher at pH 8.0 than at pH 4.0 and 6.0, and the difference was significant (except at three days post hatching). We hypothesize that changes in morphology, hatch rate, osmolality, immunity-associated gene expression, and growth rates of P. olivaceus eggs and larvae at low pH are connected to an ion-regulation defect. So, this results demonstrates that low pH level is detrimental to P. olivaceus fertilized eggs.
CONCLUSION
Many studies have examined the effects of acidification on freshwater fish species, however, there are fewer reports for marine fishes. In this study, we investigated the effects of acidic environments on P. olivaceus eggs and larvae and our results are similar to those from most corresponding freshwater fish studies. Therefore, further studies on marine fish species should be designed based on previous studies of similar effects in freshwater species. pH is one of the main environmental factors affecting aquatic organisms, along with salinity and water temperature. The observed effects on P. olivaceus eggs and larvae at low pH are assumed to be connected to ion-regulation. Future research should investigate how ion exchange is carried out by yolk sacs and chloride cells in the fertilized eggs and larvae of P. olivaceus, and calcium requirements and feeding capacity under low pH conditions.